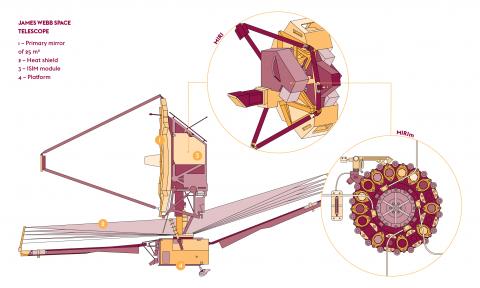
James Webb Space Telescope: when astrophysicists see (infra)red
(This article was originally published in L'Édition No.17)
The James Webb Space Telescope, with its staggering dimensions and four on-board scientific instruments, is heading for space with the promise of delivering the most exciting infrared images of the Universe.
By their own admission, the goal of astrophysicists can be summed up in a few words: to see ever more of the radiation coming from the universe and to obtain as much information from it as possible. Space telescopes play an important role in this quest. Unlike groundbased observatories, the fact that these telescopes are in space frees them from the distortion and absorption of radiation caused by the Earth’s atmosphere which severely impairs observations.
The James Webb Space Telescope (JWST) is the latest in a 25-year collaboration between the US (NASA), European (ESA) and Canadian (CSA) space agencies. Although it is the successor to the Hubble Space Telescope (HST), which was launched in 1990 to orbit the Earth and which is still in operation, the JWST also follows on from the Spitzer Space Telescope, which was launched in 2003 and has completed its mission, and with which the JWST also shares the same love for infrared radiation. While the images and spectra recorded by the HST are in the UV, visible and near-infrared range, the JWST will work in the infrared range to wavelengths of 28 microns (μm). This is because the first very distant stars and galaxies can be observed in the infrared range as their radiation has shifted towards the red end of the spectrum as a result of the expansion of the universe. This spectral range reveals the interstellar dust and gas from which new stars and planetary systems form. It also makes it easier to observe exoplanets, which are planets outside the Solar System which orbit a very bright star in the visible spectrum.
Although it has been postponed many times, the JWST is now expected to be launched after 24 December 2021 from the Guiana Space Centre in Kourou. With its 25 m2 primary mirror folded in the head of the Ariane 5 rocket fairing, it will be launched towards its future orbital position, the second Lagrangian point (L2) located 1.5 million kilometres behind the Earth in relation to the Sun. It will reach this point after four weeks. In the meantime, the JWST will deploy its huge sunshield and primary mirror. The shield, which is the size of a tennis court, will protect the telescope from radiation from the Sun, Earth and Moon and keep it as cold as possible - at -233°C or 40 K – to limit stray infrared radiation it emits. The mirror is made from 18 hexagonal panels covered with a thin film of gold which will reflect and focus the radiation to four on-board measuring instruments.
In search of the first light
Once Lagrangian point L2 has been reached and after a testing phase of several months, the telescope and the international teams of scientists will get on with the real business of studying the first stars and galaxies born more than 13 billion years ago, as well as the evolution of galaxies, stars, planetary systems and protoplanetary disks, and conditions for the appearance of life, etc.
The size of the JWST, as well as the performances of its four scientific instruments promise a wealth of discoveries, unlike any previous space observatory. Measuring 6.5 metres in diameter, the JWST has an angular resolution which is about ten times greater than that of the Spitzer Space Telescope and a hundred times greater sensitivity. Its four instruments, called NIRCam, NIRSpec, NIRISS and MIRI, are housed at the heart of the telescope in the integrated science instrument module (ISIM). The first three operate in the near-infrared range (between 0.6 and 5 μm). NIRCam is a camera and spectral imager. NIRSpec is a multi-object field integral spectrometer and NIRISS is a slitless imager and spectrometer. The MIRI (Mid-InfraRed Instrument) is a spectro-imager operating in the mid-infrared range between 5 and 28 μm.
MIRIm – an imager with three functions
MIRI, which is the result of a collaboration between the United States and Europe, is a little technological gem. It is made up of two distinct elements – a field integral and medium spectral resolution spectrometer (MRS) and an imager (MIRIm). These two sub-instruments share three infrared detectors of the same type, each with 1024 x 1024 pixels. The development of MIRIm was entrusted to a European consortium whose French contribution was led by CEA under the guidance of CNES.
The design, construction and assembly of MIRIm was carried out by the Astrophysics, Instrumentation and Modelling Paris-Saclay Laboratory (AIM – Univ. Paris-Saclay, CNRS, CEA, Univ. de Paris), with the participation of the three technical departments of the Research Institute for the Fundamental Laws of the Universe (Irfu) and three other laboratories in France - the Institute for Space Astrophysics (IAS – Univ. Paris-Saclay, CNRS) in Orsay, the Laboratory for Space Studies and Instrumentation in Astrophysics (LESIA – Observatoire de Paris, CNRS, Sorbonne Univ., Univ. de Paris) in Meudon and the Marseille Astrophysics Laboratory (LAM – CNRS, CNES, Aix-Marseille Univ.) –, as well as partners in Europe.
MIRIm provides three observing modes: an imager mode to photograph space through nine wide-band mid-infrared filters, a low-spectral resolution spectrograph mode to split light into spectra and identify the chemical elements and molecules observed, and a coronagraph mode to mask the light of a star and observe its close environment.
An innovative design
AIM and IAS have been involved in the project right from the start. The significant amount of responsibility taken on by these laboratories is due to the scientific and technical expertise they acquired during their participation in previous long-wave space missions (ISO, Herschel, Planck, etc.). However, developing an instrument such as MIRI was still a challenge in 1998. This is because this instrument, unlike the others in the JWST, has to be actively cooled to function optimally. “MIRI must reach below 20 K and its infrared detectors must even be at a temperature of 7 K (-266 °C), otherwise a dark current limits observations. Using a liquid helium tank for cooling, as was the case with previous infrared space instruments, would have limited MIRI’s lifetime to a few years,” says Pierre-Olivier Lagage, a researcher at the AIM laboratory and principal investigator (PI) for MIRIm. The active cooling system developed for MIRI, based on closed-cycle machines, is a real technical feat.
“The optical design of MIRIm began in 1998, but it took us five years to construct the instrument’s design because it had to be compact and have only one mechanism. It took us some time to find the solution, which was based on a single filter wheel which was compatible with the three observation modes of MIRIm,” adds Pierre- Olivier Lagage. This wheel has nine spectral filters. These different filters allow radiation to pass through within a given range of wavelengths. “By rotating the wheel in sequence so that each filter is positioned in front of the detector, a set of multispectral images is obtained,” explains Alain Abergel from IAS.
IAS was responsible for designing and developing the telescope’s simulator used for MIRIm optical tests carried out in the AIM laboratory. “Once the instrument was built, its optical features had to be checked to ensure they corresponded to the specifications before delivery,” says Alain Abergel. The team at the AIM laboratory built the test bench comprising a vacuum chamber equipped with a cryostat and a simulator from the JWST. “That was an important step in the project. There was no room for error as once Lagrangian point L2 has been reached, nobody can go and repair the telescope if it needs it!” points out Pierre-Olivier Lagage.
Improving the raw data
In addition to MIRIm developments, the researchers developed innovative algorithmic methods to improve the data produced. This is because any instrument ‘degrades’ the objects observed, either spatially or spectrally, or by adding noise. “Ideally, the observation of a distant star should give a point. However, in practice, more often than not it results in a larger spot. This is called the point spread function,” explains Pierre-Olivier Lagage. Fundamental in astrophysics, this function stems directly from the physical phenomenon inherent in any camera - i.e. the diffraction of the incident ray at the entrance to the lens. “The larger the telescope, the smaller the spot becomes,” says Pierre-Olivier Lagage. Despite its 6.5 metre diameter and much higher spatial resolution than its predecessors, the ability of the JWST to separate very close points in the sky is still limited. “The image obtained is never perfect. The spatial information is degraded and this is true for any optical instrument,” concludes Alain Abergel. As result, data has to be “unblurred”.
The correction of ‘defective’ pixels from the infrared detectors was another factor to take into consideration. It is statistically proven that a very small number of pixels will not function nominally in each detector. They are much like dead pixels, which remain black in the image, or those affected by cosmic rays which produce outliers. To correct this, the technique of ‘dithering’ is used. This consists of obtaining several images of the same region slightly offset from each other. “We make sure that several different pixels, at least one of which is not ‘defective’, observe the same area,” explains Alain Abergel. By shifting the images very slightly between each shot, superresolution is also achieved, which improves the spatial information contained and results in a sharper image.
Exoplanets in the sights
If there is one area of research which has grown over the years, it is that of exoplanets. The first exoplanet was discovered and observed in 1995. Many others subsequently then followed (there are now more than 4,700 of them and their number is growing), so using the JWST to better identify them quickly became an obvious thing to do. The European consortium, which as constructor of the MIRIm is guaranteed 450 hours of observation, will devote 110 hours to the study of exoplanets. The others programmes will look at protoplanetary disks of dust, extragalactic observations, supernova 1997A and photodissociation regions.
The JWST will focus on writing a new chapter focused on the analysis of the atmosphere of exoplanets. The detection of these planets can be done indirectly thanks to the small variation in the luminosity of a star which they cause when they pass in front of it. However, these variations are sometimes so weak that many other effects dominate and noise overpowers the signal to the detector. The MIRIm’s phase mask coronograph, whose technology was developed at the Meudon Observatory, allows the direct detection of objects in the vicinity of the star by ‘turning off’ the star itself.
Scientists are looking to see the emission spectrum of these planets to analyse the composition of their atmosphere. “When the planet passes in front of its star on the telescope’s line of sight, if it has an atmosphere and molecules are present, these stop the light emitted by the star in distinctive spectral bands which can be identified by the spectrograph,” explains Pierre- Olivier Lagage. These molecules, such as water, methane, carbon monoxide or dioxide, phosphine, etc. all have infrared signatures.
“The problem is that the James Webb Space Telescope was originally designed to see into the far distance. However, as the observed stars are close, their radiation saturates the images. As a result, a slitless spectroscopy mode has been added to MIRI which reads only a part of the detector,” says Pierre-Olivier Lagage. “In the end, the delay in the launch of JWST was used to secure the best methods for analysing the data and to define the best targets to study.”
The JWST will be focusing its instruments largely towards the planet TRAPPIST-1 b which orbits its star TRAPPIST-1. This star, located in the Aquarius constellation which is about 40.5 light years from Earth (nearly 4.1014 km) has a planetary system of at least seven rocky exoplanets. Several of them are in the habitable area around the star, which means water could be present there in a liquid state. “TRAPPIST-1 b is the closest planet to the star and has a temperature of 400 K,” says Pierre-Olivier Lagage. But will it be possible to detect biosignatures (those famous signs of the existence of life) on an exoplanet? “The biosignatures could be very different from what we think of today,” points out Pierre-Olivier Lagage. “What is certain is that we’ll be ready if we observe something abnormal. The range of discoveries made in recent years on exoplanets is such that no matter how many models we have, we are sure that there will be surprises.”
A centre of expertise for the community
The MICE centre of expertise created by AIM, IAS, LESIA and LAM and supported by CNES aims to make the knowledge acquired by these teams about MIRI available to the scientific community. “Through a project such as the JWST, the teams working on it develop a genuine expertise with the instrument. This should not be lost once the project is delivered, but should instead be used for other purposes,” says Alain Abergel. “The centre will also develop sophisticated methods to improve the data analysis,” concludes Pierre-Olivier Lagage. “The best multispectral images from MIRIm will be delivered to astrophysicists,” adds Alain Abergel.
The INCLASS joint laboratory set up between IAS and PME ACRI-ST based in Sophie Antipolis specialises in the observation of Earth from space and the development of ground segments. This laboratory will bring complementary skills to the centre of expertise. “Space-based Earth observations face data treatment problems comparable to those of the JWST,” explains Alain Abergel. The LabCom, created at the start of May 2021 and which has funding from the ANR lasting four years, aims to develop innovative algorithmic methods for merging imaging and spectroscopy data. “In particular, we hope to extrapolate the spectroscopic information obtained on a small region of the sky by the MRS spectrometer to the wide-field images from MIRIm,” explains Alain Abergel.
For the time being, scientists are eagerly awaiting the ‘in-flight calibration’, or the first tests in real conditions, and then the first astrophysical observations, which should begin by June 2022. The JWST is scheduled to operate for five years (but everyone hopes for twice that) and will be THE large space observatory available to astrophysicists around the world. “The purpose of astronomical observations is to advance our physical understanding of the Universe, and with the JWST we will enter a new world. It’s not just going to be a little better – it will be a hundred times better! The images and spectra provided will be of unprecedented quality and richness. Their interpretation will require the development of highly detailed physical models. That will take years,” predicts Alain Abergel.
Publications
- M. A. Hadj-Youcef et al., Fast Joint Multiband Reconstruction From Wideband Images Based on Low-Rank Approximation, IEEE Transactions on Computational Imaging, vol. 6, pp. 922-933, 2020.
- O. Venot et al., Global Chemistry and Thermal Structure Models for the Hot Jupiter WASP-43b and Predictions for JWST. The Astrophysical Journal, vol. 890, 176, 2020.
- P. Bouchet et al., The Mid-Infrared Instrument for the James Webb Space Telescope, III: MIRIM, The MIRI Imager. Publications of the Astronomical Society of the Pacific, vol. 127, 953, 2015
- https://jwst.nasa.gov/content/webbLaunch/index. html