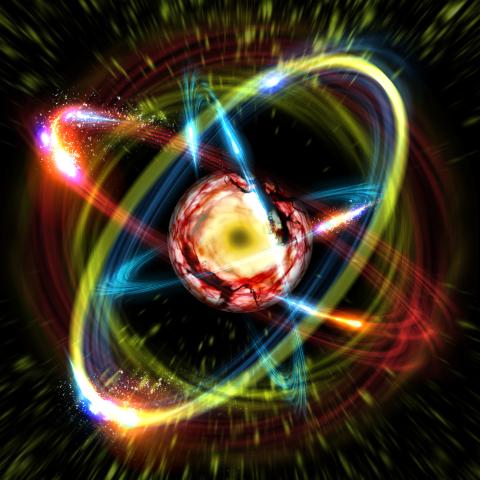
In search of antimatter
This article was originally published in L'Édition n°23.
Antimatter. This mysterious term, behind which lies a physical concept that first appeared in the early 20th century, has inspired numerous science-fiction writers and fuelled their wildest literary fantasies: fuel for spaceships for some, a delusion of power or a weapon of mass destruction for others. But from a scientific point of view, what is antimatter and where does it come from? Scientists are working on answering these questions through research carried out in large particle accelerators, going all the way back to the very first moments of the Big Bang. Particles created when energy is transformed into matter, antimatter also complements the matter needed to create the vacuum.
To trace the history of antimatter and its beginnings, we have to go back almost a century, to 1928, when Paul Dirac, a British physicist and winner of the Nobel Prize for Physics in 1933, wished to integrate the principles of the theory of special relativity, drawn up by Albert Einstein, into quantum physics. Introducing the notion of space-time, the link between energy and the mass of an object, and the speed of light in a vacuum as an invariant quantity, special relativity and general relativity, focused on gravitation, all describe in the form of equations the behaviour of matter and its objects on an infinitely large scale.
But by applying these mathematical formulas to the infinitely small, Paul Dirac obtained two different solutions to his equations. While the first solution is well known and relates to the electron, a negatively charged particle smaller than the atom of which it is one of the constituents, along with protons and neutrons, whose existence was first postulated in 1897 by the British physicist Sir Joseph John Thomson and confirmed in 1911 by Ernest Rutherford, this is not the case for the second solution. The particle to be associated with it has the same mass and the same spin as the electron, but its charge is the opposite. The physicist then introduced the theoretical notion of the antielectron. Four years later, Carl David Anderson first observed this famous antiparticle in his fog chambers and named it the positron. Antimatter was no longer a theorist’s whim; it really does exist.
Since then, study on antimatter has continued and other antiparticles have been produced experimentally, such as antiprotons. Finally, for every particle of matter, there is a partner antiparticle, with an identical mass and spin but opposite quantum numbers, including the electrical charge. Similarly, an antiatom is an atom made up of elementary antiparticles: its nucleus contains antiprotons and antineutrons, around which positrons gravitate. This is the case, for example, with antihydrogen, formed from an antiproton and a positron. There is, however, one exception: the photon. This quantum of energy associated with light is the only one to be both an elementary particle and at the same time its antiparticle.
Complementing material to create a vacuum
But the most original aspect is yet to come. When a particle comes into contact with its antiparticle, an annihilation reaction occurs: both particles disappear, and energy is produced. It is easy to calculate the value of this energy using Albert Einstein’s well-known relationship: E=mc², where "E" is energy, "m" the mass of the particle and "c" the speed of light in a vacuum. However, this reaction is reversible: it is possible to produce matter from energy alone. The result is inevitably a particle-antiparticle pair. Scientists believe that this is what happened at the time of the Big Bang; the immense amount of energy released would have created as much matter as antimatter. However, two major questions remain: where is the created antimatter now, given that the Universe is essentially made up of matter, and why didn’t matter and antimatter annihilate each other immediately after they were created? With the exception of a few positrons produced naturally by cosmic rays, we have never yet succeeded in observing natural antimatter in the Universe.
So where does antimatter hide?
Scientists are currently investigating two possible explanations for the disappearance of antimatter. The first imagines the emergence of an initial asymmetry at the moment of the Big Bang, resulting in an excess of matter. In this way, all of the minority antimatter would have been annihilated along with the vast majority of matter, leaving only the excess of the latter in the Universe. However, the very large amount of matter still present in the Universe makes this hypothesis difficult to corroborate in the current state of scientific knowledge, which does not allow us to understand the origin of an initial asymmetry of this magnitude.
The second hypothesis is that of a possible repulsion between matter and antimatter. This repulsion would have created two distinct zones in the Universe, one made of matter and the other of antimatter. But if this had been the case, somewhere in the Universe there would be a boundary between the two zones, where particles and antiparticles would come into contact. At this point, their annihilation should have produced a very specific type of radiation, detectable by the tools and equipment available to scientists today. So far, however, none of them has succeeded in observing such radiation in the Universe.
How can antimatter be produced and studied?
To confront these two hypotheses, physicists are now studying the differences in behaviour between matter and antimatter. This implies successfully producing antimatter beforehand. This is done in very powerful particle accelerators. In Europe, these experiments take place mainly at CERN (originally the European Council for Nuclear Research, now the European Laboratory for Particle Physics), on the French-Swiss border.
CERN is the world’s largest particle physics centre, with a Large Hadron Collider (LHC) 27 km in circumference. A colossal network of superconducting electromagnets guides protons through this gigantic particle accelerator, which operates in a high vacuum similar to that in outer space, almost at the speed of light. Some protons rotate in one direction, others in the opposite. These two groups of protons then collide at four contact points, all equipped with state-of-the-art detectors. As Marie-Hélène Schune, director of research at the Laboratory of the Physics of the Two Infinities - Irène Joliot-Curie (IJCLab - Univ. Paris-Saclay, CNRS, Univ. Paris Cité), who is particularly involved in the LHC experiments, says: "At the moment of collision, protons must be considered as reservoirs of energy." In fact, a beam of protons launched at this speed stores an energy of 360 MJ, equivalent to that of a TGV at 150 km/h, although such a beam represents only one millionth of a gram of matter. More unstable antiparticles are produced locally at collision points, and because of their instability, they are studied directly by the detectors around the LHC. Some of the protons produced in the pre-accelerator are not sent to the LHC, but to a target point where they generate sufficient energy to create matter/ antimatter pairs. The antiprotons created are then redirected to the antiproton decelerator (AD), where powerful magnets begin to slow them down before they enter the 10 m diameter ELENA (Extremely Low Energy Antiproton) ring, which further contributes to their deceleration. Antiprotons produced and slowed down in this way are easier to handle and analyse. The facility distributes them to the various research teams who study them or use them to produce antihydrogen atoms.
The Standard Model of particle physics
The Standard Model of particle physics is a theory which was drawn up in the second half of the 20th century by physicists from all over the world, and which describes the organisation of matter at an infinitely small scale and explains the origin of phenomena observed at this scale. The theory, progressively confirmed and updated by experiments, introduces the existence of elementary particles of two different types, fermions and bosons. All these particles are characterised by a mass (in electronvolt/c2 or eV/c2), a spin (angular momentum) and sometimes a particular colour, which is actually a colour in name only, and an electric charge (positive or negative).
Fermions are the elementary particles of matter. They are divided into two different groups, quarks and leptons, each with six representatives, and grouped in pairs or generations. The first generation of fermions contains the lightest, most stable particles that make up ordinary matter. The second and third generations contain the heavier, unstable particles, which rapidly disintegrate into first-generation particles. Quarks do not exist in isolation, but rather come together to form composite particles known as hadrons. Gathered in specific trios, quarks constitute baryons, in which we find, for example, the proton and the neutron. Special quark/antiquark duos give rise to mesons, such as the pion or the kaon.
Bosons, on the other hand, are elementary particles that produce effects on particles of matter. There are two different types of boson: interaction vector bosons and a mass boson. Vector bosons carry three of the four fundamental particle interactions (or forces): strong, weak and electromagnetic. The fourth fundamental interaction - gravitational - cannot be explained by the Standard Model, but is negligible at the scale of elementary particles, as their mass is extremely small. Gluons carry the strong interaction, which depends on particle colour, and are found inside hadrons to hold quarks and antiquarks together. The photon is the vector of the electromagnetic interaction, which depends on the electrical charge of the particles and enables electrons (negative) to be linked to a nucleus formed of protons (positive) and neutrons (neutral) to form atoms. The Z and W bosons carry the weak interaction responsible for the radioactive decay of atomic nuclei. The Higgs boson, whose existence was confirmed just over a decade ago, confers mass on the two previous bosons and fermions.
Antimatter: does it fall?
As part of the Gravitational Behaviour of Antihydrogen at Rest (GBAR) experiment at CERN, involving Yves Sacquin and a team from the Department of Particle Physics (DPhP - Univ. Paris-Saclay, CEA), scientists are seeking to discover whether gravity has the same effect on matter as on antimatter; in other words, do matter and antimatter "fall" in the same way? Because in the case of inverted gravity, i.e. if antiparticles were to "fall" upwards, this would suggest that matter and antimatter repelled each other during the Big Bang, thus avoiding total annihilation.
The GBAR experiment at CERN aims to observe and study the fall of antihydrogen atoms subjected to gravitational force in a vacuum, as a hydrogen atom is composed of an antiproton and a positron. These antiatoms are produced in several different stages. Antiprotons are generated in the Centre’s large particle accelerators and slowed down by the ELENA facility, while positrons are produced by the impact of a stream of electrons on a target made of tungsten, an extremely hard and dense metal. From the positrons formed, scientists produce atoms of positronium, a bound and highly unstable state consisting of an electron and a positron. Positroniums are produced by a positron flux directed onto a positron/positonium converter, in this case a thin mesoporous silica membrane, giving rise to a cloud of positroniums. Antiprotons are fed into this chamber at CERN, where they collide with a positronium whose positron gravitates around the antiproton, producing atoms of antihydrogen. However, the gravitational force exerted on these very light atoms is too weak in relation to their kinetic energy to see them fall directly. We have to slow them down, which is a real challenge.
Producing hydrogen anti-ions
To achieve this, GBAR scientists plan to produce ionised antihydrogen atoms, i.e. anti-ions comprising an antiproton accompanied by two positrons, represented as pronounced "H bar plus"; the bar is the sign of antimatter in particle physics). As they are positively charged, these anti-ions are easier to manipulate and cool down to sub-Kelvin temperatures, using lasers and Be+ ion crystals. After cooling them in a laser field, the scientists capture them in a second trap. The anti-ions are kept immobile, and then, by removing a positron with a laser, antihydrogen atoms with virtually no kinetic energy are obtained. No longer ionised, these antiatoms are no longer held by the trap and are subject to gravity. Detectors then record their annihilation at the end of their fall. By measuring their fall times and impact locations, scientists hope to determine the effect of gravitational interaction on antimatter.
Although GBAR scientists have not yet succeeded in producing anti-ions , they have developed a technique for generating an intense flow of positrons, and have produced their first atoms of antihydrogen, a necessary step not only for the formation of anti-ions but also for other applications. In fact, this innovative technique is the basis of the Posithôt start-up, a spin-off from the Department of Particle Physics (DPhP - Univ. Paris-Saclay, CNRS, CEA). The company provides quality control of materials using positron flux (see this issue’s Business & Innovation section).
A first idea of the fall
Although GBAR has yet to provide an answer to the question of how antimatter falls, ALPHA, another experiment at CERN, is already providing encouraging initial results: according to these results, hydrogen antiatoms do fall downwards. But even if we know that gravity is not reversed for antimatter, its effects are not yet precisely known. The antihydrogen atoms used in the ALPHA experiment are not sufficiently cooled to ensure that the effects of gravity are identical on matter and antimatter. Observing such a difference would demonstrate the inaccuracy of Einstein’s equivalence principle, which states that the gravitational force acts on objects independently of their composition and internal structure. For the time being, GBAR scientists are continuing their efforts, hoping one day to discover any possible differences in behaviour between matter and antimatter.
Publications :
- M.Charlton et al., Positron production using a 9 MeV electron linac for the GBAR experiment, Nuclear Inst. And Methods in Physics Research, 985, 164657 (2021).
- P. Adrich et al., Production of antihydrogen atoms by 6 keV antiprotons through a positronium cloud, Eur. Phys. C. C83, 1004 (2023).
Matter and antimatter: is there an asymmetry?
What if the asymmetry between matter and antimatter observed in the Universe came from the very moment of its creation? This is what Marie-Hélène Schune and her team at the Laboratory of the Physics of the Two Infinities - Irène Joliot-Curie (IJCLab - Univ. Paris-Saclay, CNRS, CEA, Univ. Paris Cité) are trying to find out within the framework of the collaboration with the LHCb (Large Hadron Collider beauty) at CERN. This experiment focuses on the potential differences in behaviour between matter and antimatter, and more specifically on a phenomenon known as CP symmetry violation on a b quark (known as beauty quarks).
CP symmetry is a dual principle according to which the same results would be obtained between an experiment performed with matter particles and its mirror experiment, performed with the corresponding antimatter antiparticles. C symmetry corresponds to an inversion of the particle’s electrostatic charge and other quantum numbers, such as colour. P symmetry, known as parity symmetry, acts on the particle’s configuration, like a mirror in which left becomes right. If there is no difference in the behaviour of matter and antimatter, CP symmetry is preserved. Otherwise, CP symmetry is violated.
As far as we know, the powerful electromagnetic interactions are conserved under CP transformation. However, this does not appear to be the case for the weak interaction.
Tracking the decay of beauty with LHCb
To confirm this, the LHCb experiment at CERN’s large LHC particle accelerator is studying CP symmetry violation by the weak interaction in the decay of hadrons, composite particles made up of quarks. The ones scientists are most interested in contain a b quark (known as beauty quarks), for which, according to the theory, the CP symmetry violation would be the most visible.
It is at the LHCb detector that protons launched at almost the speed of light collide. The result of this collision and the energy released are hadron pairs B+ and B-. B+ consists of a b antiquark and a u quark, while B- combines a b quark and a u antiquark. These hadrons are particularly unstable. "In 10-12 seconds, they disintegrate, giving rise to other particles that are stable," explains Marie-Hélène Schune. These more stable particles are then perceived by the LHCb detector and their quantity is measured very precisely.
Formed from energy, B+ particles and B- antiparticles must logically be present in equal quantities. However, if the weak interaction does not violate CP symmetry, i.e. if matter and antimatter behave in the same way, the decay products of B+ and B- should also be detected in equal quantities. However, in 2021, scientists observed a significant difference of over 13% between the two decay reaction products, representing material evidence of CP symmetry violation. The IJCLab researcher confirms: "The fact that CP symmetry is violated by the weak interaction means that there is a difference in behaviour between matter and antimatter." Since the LHCb experiment began collecting data, over 70 scientific papers have been published on the measurement of CP violation.
If these results highlight a difference in behaviour between matter and antimatter, scientists still have to study where this difference comes from, and whether other violations have so far eluded them. New research has already begun...
Publication :
- The LHCb collaboration, R. Aaij et al. Measurement of CP observables in B± → D(*)K± and B± → D(*)π± decays using two-body D final states, JHEP 04, 81 (2021).
This article was originally published in L'Edition No. 23.
Find out more about the journal in digital version here.
Subscribe to the newsletter and never miss an issue.