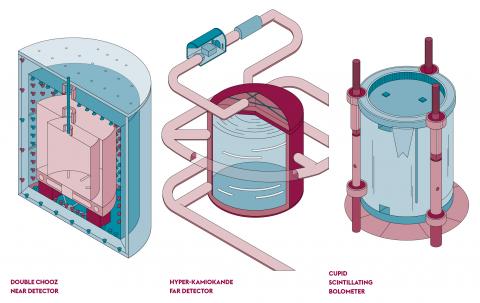
The neutrino: towards physics and beyond
(This article was originally published in L'Édition n°16)
Thanks to large-scale experiments, physicists at Université Paris-Saclay are working to unravel the mysteries surrounding the neutrino and are looking ahead to a new model of particle physics.
Developed in the mid-1970s and extended since then, the Standard Model is the centrepiece used in particle physics to explain observable phenomena at this scale and to understand the structure of matter. Based on discoveries made over 100 years ago, this theoretical model organises and describes the elementary building blocks (fermions) that constitute matter, and the fundamental interaction modes that govern them. It distinguishes 12 fermions – six quarks and six leptons and their antiparticles of the same mass but opposite charge – divided into three families, and bosons that orchestrate three of the four fundamental forces of the universe – strong, weak and electromagnetic.
Although the majority of the experimental results still coincide with its theoretical principles, some imperfections are apparent. And for several years, researchers have been pursuing a new physics, beyond the standard model. In their quest, they are interested in the neutrino, an elementary lepton that is still very enigmatic but improved knowledge about it promises to revolutionise our perception of the universe.
With a neutral charge and a mass 465,000 times less than that of the electron, the neutrino fascinates by its ghostly singularity: it interacts very little with matter, one million billion times less than the electron, and only by the weak force. This explains why it has remained under the radar for a long time. Generated massively at the heart of the Sun, during cosmic cataclysms or by cosmic rays in the atmosphere, neutrinos travel without making a ripple and every second, 65 billion of them pass through every square inch of the Earth and its inhabitants, like a wall pass.
Neutrinos in three flavours
Considered as early as 1930 by the physicists Wolfgang Pauli and James Chadwick, their existence was proven in 1956 by the Americans Frederick Reines and Clyde Cowan, who observed them for the first time in the radiation emitted by a nuclear reactor: during nuclear fission, a uranium nucleus breaks into two smaller, unstable nuclei, which return to stability by emitting either photons – this is gamma radioactivity – or electrons and neutrinos – this is beta (β) decay with neutrino emission. “These are antineutrinos, the antiparticles of neutrinos, of electronic type, or ‘flavour’, as they are emitted with their lepton partner, the electron,” Matthieu Vivier explains, a researcher at the Particle Physics Division (DPhP – Univ. Paris-Saclay, CEA) of the Institute of Research into the Fundamental Laws of the Universe (IRFU).
Together with protons and neutrons – trios of up and down quarks – and electrons, electron neutrinos constitute the first family of fermions. In 1962, and again in 2001, experimental observations revealed two other neutrino flavours that add to two families of fermions: the muon neutrino, the companion of the muon, and the tau neutrino, the companion of the tau. A neutrino flavour is in fact a superposition of three mass states marked 1, 2 and 3.
But more surprisingly, the neutrino is capable of changing its flavour during its propagation. A phenomenon called neutrino oscillation and which “relies on the hierarchy of mass states, which do not propagate at the same speed, and the mixing angles Θ12, Θ23, Θ13, which govern the probability of a neutrino changing flavour,” explains Fabien Cavalier, a researcher at the Irène-Joliot Curie Physics of Two Infinities Lab (IJCLab – Univ. Paris-Saclay, CNRS, Univ. de Paris). This oscillation is the focus of many international experiments.
Swinging from the reactor angle
Nuclear reactors are the medium of choice for its study. The fission rate is such that a reactor produces between 1020 and 1021 electron antineutrinos every second. There is hope for “some” interaction with the surrounding matter. To detect this, scientists use inverse β decay. “It is the capture of an electron antineutrino by a proton. This produces a neutron and a positron, the antiparticle of the electron. If during the experiment we detect two concomitant signals characteristic of these two particles, this signals the reaction and distinguishes it from the ambient background noise due to natural radioactivity and cosmic rays,” Matthieu Vivier explains.
This is the principle used by the Double Chooz experiment, set up in 2006 at the Chooz power plant in the Ardennes (France) and which recently delivered its last results before being dismantled. Double Chooz was designed to precisely measure the oscillation mode associated with the parameter Θ13, which reflects a deficit of electron antineutrinos, which become muonic or tauic at a certain distance. “In the case of reactor antineutrinos, whose energies reach the MeV, this oscillation mode develops at about 1 km,” Matthieu Vivier comments, who worked on Double Chooz. Located 400 m from the reactor in an underground cavity, a first detector measured the number of electron antineutrinos emitted and a second detector 1,500 m away, measured those still present further away. “In the end, about 5% of neutrinos change flavour.”
Inside the detectors, transparent tanks filled with a sparkling liquid (a kind of mineral oil) rich in protons and to which a chemical compound had been added, were responsible for collecting the signal. “When a neutrino passes through the liquid, it is captured by one of the protons present, and the neutron and positron then emitted deposit their energy in the liquid, which re-emits it in the form of scintillation light,” Matthieu Vivier says. At Double Chooz, nearly 400 immersed photomultipliers recorded and then translated this light into electronic signals that scientists could interpret. They were able to measure the mixing angle Θ13 with an accuracy of about 10% and show that it is not zero.
“With Double Chooz, we also reviewed the calculations for predicting neutrino fluxes and re- alised that previous experiments had observed neutrino rates at very short distances from the reactors that were lower than predicted,” Matthieu Vivier reports. An anomaly that gave rise to a postulate: the existence of a new oscillation mode and a fourth, sterile and non-reactive type of neutrino. This hypothesis was recently swept aside by the results of the STEREO experiment conducted a few dozen metres from the reactor of the Laue Langevin Institute in Grenoble. Operating on the same principle as Double Chooz, the detector is on the way to excluding the existence of a sterile neutrino, “with a good degree of confidence”. “To explain this anomaly, we would have to look at the theoretical models and check whether there is any calculation bias in the estimate of the neutrino fluxes emitted by the reactors,” Matthieu Vivier says.
Detectors with new technologies
For the time being, he and his colleagues are participating in the design of a new experiment, Nucleus, which will be set up in Chooz, 50-100 m from the reactors. It aims to study another neutrino interaction process: coherent scattering. With a probability of occurrence almost 1,000 times greater than inverse β decay, it would open up the exploration of very low energy regimes, from 10 to 100 eV. “Nucleus does not use the same detection techniques – there is no sparkling liquid – because the energies involved are far too low.” The experiment uses bolometric detectors composed of cryostat-cooled sapphire and calcium tungstate crystals. “When an antineutrino hits a nucleus in the crystal, the nucleus recoils under the ‘shock’ and a fraction of the energy is deposited in the crystal, which re-emits it as heat.” The slightest increase in temperature is then measured by an ultrasensitive thermometer. “This is a tungsten film placed at a transition temperature between a normal and superconducting regime. The measurement of a voltage change across the thermometer indicates a deposition of energy in the detector.” The team also hopes to reduce the size of the detectors. Nucleus’ will total 10 g. A real technological breakthrough.
Also at Chooz, the IJCLab plans to install a new concept of neutrino detectors. With the LiquidO project, developed by Anatael Cabrera and his colleagues, the idea is to dispense with transparent, sparkling liquid in favour of an opaque, glittering liquid. “The detector is filled with tightly packed optical fibres that collect the emitted photons of light. The first results are very conclusive and prototyping is underway,” says Fabien Cavalier.
The question of matter / antimatter asymmetry
The IJCLab and Irfu teams are also heavily involved in the next generation of experiments to measure the oscillations of neutrinos produced by particle accelerators: DUNE in the United States and Hyper-Kamiokande, the big sister of the current T2K experiment in Japan. These experiments address another major flaw in the Standard Model: the matter/ antimatter asymmetry of the universe. “The widely held assumption is that in the Big Bang, both matter and antimatter were created. So everything should have annihilated each other. In this case, how can we explain the fact that there is so much matter left in the Universe and that all the antimatter has disappeared?” says Sandrine Emery-Schrenk, researcher at the DPhP.
This paradox has led the teams to analyse and compare the behaviour of particles and antiparticles, and to tackle another major paradigm: CPT symmetry. This combination of three components – charge (C), parity (P), time (T) – mathematically describes the behaviour of matter and antimatter. “This is a strong theorem in particle physics. It says that if you reverse the charge and parity, an antiparticle going backwards in time would behave like a particle going forwards. Mathematically, the behaviours should follow the same equations,” Fabien Cavalier explains. Established for quarks, this CP symmetry violation is however not sufficient to explain the matter/ antimatter asymmetry, and DUNE and Hyper- Kamiokande will have to confirm the existence of a CP symmetry violation for neutrinos, widely suspected by the scientific community.
Accelerating particles to accelerate discovery
As in the case of neutrinos from nuclear reactors, these new experiments use two types of detectors, near and far. They are based on the results being accumulated by T2K and its distant detector Super-Kamiokande, located underground 295 km from the source, and which will begin its second phase at the end of 2022.
In principle, protons, accelerated to very high energies (30 GeV), strike a carbon target and break the nucleons of the material. This releases a cascade of particles, including pions selected in a tunnel with the help of magnetic horns. These decay into muon neutrinos or antineutrinos that are sent in a beam to the far detector, and it is their transformation into electron neutrinos and antineutrinos that is there measured. “By adjusting the charge of the pions, we generate either a beam of neutrinos or a beam of antineutrinos,” Sandrine Emery-Schrenk explains. At the finish line, a tank containing tons of perfectly transparent liquid water and adorned with photomultipliers records the blue light emitted by the passage of muons and electrons from the quasi-elastic interactions of neutrinos and traces them back to their flavour.
If matter is perfectly symmetrical, the probabilities of a neutrino and its antiparticle, the antineutrino, changing flavour on the way must be the same. However, the T2K results seem to indicate the opposite: the probability is much higher in the case of a neutrino than an antineutrino. The aim of T2K phase II and the future DUNE and Hyper-kamiokande experiments is to enrich the data sets and improve the statistics. “The challenge is to bombard the target with enough protons to produce a more intense beam of neutrinos,” Fabien Cavalier explains, who is involved with the IJCLab in the creation of the DUNE proton accelerator at Fermilab. “Thanks to these much more powerful neutrino beams and more massive far-field detectors, we should be able to triple the amount of data collected by T2K phase II, and even more by Hyper-Kamiokande”, says Sandrine Emery- Schrenk, who is involved in the development of the T2K Phase II instrumentation, including the near detector that will also be useful for Hyper-Kamiokande and DUNE.
Scheduled to start up in 2026 and 2027 respectively and provide their first analyses by 2030, DUNE and Hyper-Kamiokande are gigantic in scale. If the technique used by Hyper- Kamiokande is similar to that of Super- Kamiokande, the volume of water in the far detector will be ten times greater. DUNE will contain 40,000 tonnes of liquid argon, not water. It will be located underground 1,300 km from Fermilab. With its four rectangular modules cooled to -185 °C and each containing nearly 18 000 tonnes of liquid argon, it will be the largest detector of its kind in the world.
“Each module is a drift chamber with two anodes placed around a cathode at high electrical potential. When a neutrino hits an argon nucleus, it creates an electron or a muon. These charged particles will ionise the argon, and the ionised particles produced will drift between the anode and cathode and then be collected on a grid at the top or bottom of the module. The light emitted during ionisation will be detected by photomultipliers placed around the detector. The whole system will make it possible to reconstruct the trajectory of the charged particles and to reveal the neutrinos and their flavour,” explains Fabien Cavalier, whose laboratory is responsible for the 800 m2 of glass fibre cathode and the 100 or so stacks for lowering the electronics into the cryostats.
In search of a decisive disintegration
In their search for explanations for the matter/ antimatter asymmetry, scientists are also fascinated by a very particular, rare and so far unobserved mode of decay: double β decay without neutrino emission. Great experiments are dedicated to it, as CUORE or NEMO, or in the future CUPID and SUPERNEMO, their continuation. “If we could observe it, it would tell us that neutrinos are Majorana fermions,” explains Claudia Nones, a researcher at DPhP. According to the Standard Model, for every particle there is its exact opposite, the antiparticle. If it turned out that the neutrino was both its own particle and its own antiparticle, it would elucidate the disappearance of antimatter from the universe.
But observing this decay is not easy: it occurs in a nucleus less than once every 1026 years! And only 35 natural isotopes are involved, few of which have been studied by scientists. To get away from natural radioactivity and increase their chances of seeing this decay, scientists descend into underground laboratories, such as the one in Modane in Savoie (France), where the rock forms a screen.
As a prelude to the CUPID experiment, scheduled to start in 2026 at the Gran Sasso national laboratory (Italy) and last for ten years, the CUPID-Mo experiment recently carried out in Modane is based on cryogenic scintillation bolometers. “The crystals used contain an isotope of interest. When a particle passes through one of them, it releases energy and the crystal lattice starts to vibrate. This produces phonons which increase the temperature of the crystal. This increase is then detected by a thermometer glued to the crystal: to increase sensitivity, we work at temperatures of 10 to 20 mK,” Claudia Nones explains. The bolometer is also a scintillator: when the particle hits the crystal, it emits light, which is recorded by a second type of bolometer. “A thermal signal and a light output are obtained in coincidence, to better distinguish the original particle.” While some experiments favour tellurium oxide (TeO2) crystals – the 130Te isotope being naturally very abundant (34% of natural tellurium), CUPID-Mo uses lithium molybdate (Li2MoO4) crystals, the 100Mo isotope, although less abundant (10% of natural molybdene), being more promising.
The team managed to determine the half-life of the 100Mo with the best limit in the world – 1.4×1024 years – and to initiate a change of scale with the best possible prospects. CUPID’s cryogenic scintillation bolometers will house over a thousand Li2MoO4 crystals, with a maximised arrangement. “We expect to see one event per year and per detector tonne,” Claudia Nones says.
New physics is not far off...
Publications
- The Double Chooz Collaboration. Double Chooz θ13 measurement via total neutron capture detection. Nat. Phys. 16, 558–564 (2020).
- The T2K Collaboration. Constraint on the matter– antimatter symmetry-violating phase in neutrino oscillations. Nature 580, 339–344 (2020).
- Abi, B. et al. Prospects for beyond the Standard Model physics searches at the Deep Underground Neutrino Experiment. Eur. Phys. J. C. 81, 322 (2021).
- The CUPID collaboration, Characterization of cubic Li2100MoO4 crystals for the CUPID experiment, Eur. Phys. J. C. 81, 104 (2021).